Research - (2022) Volume 10, Issue 8
Oxidative Stress and Inflammation Markers in Lipopolysaccharide (LPS)-Induced Acute Kidney Injury in RatsâThe Potential Role of Lacosamide
Rahime Aslankoc1, Ozlem Ozmen2, Oguzhan Kavrik1 and Arzu Yalcin1*
*Correspondence: Arzu Yalcin, Süleyman Demirel University, Faculty of Medicine, Department of Physiology, Isparta, Turkey, Email:
Abstract
Background: Sepsis is a well-known cause of multiple organ failure although its molecular mechanism is not fully defined. In this study, antioxidant, and anti-inflammatory effects of lacosamide (LCM) on the kidney tissue of rats with sepsis were investigated in the experimental LPS-induced sepsis model. Materials and Methods: Twenty-four female Wistar albino rats were divided into three groups: Control group, LPS group (5 mg/kg LPS, i.p, single dose), and LPS+LCM (5 mg/kg LPS, i.p, single dose + 40 mg/kg LCM, i.p, 3 days pretreatment). Malondialdehyde (MDA), superoxide dismutase (SOD) and catalase (CAT) activities were analyzed in kidney tissues. Creatinine, blood urea nitrogen (BUN) and uric acid levels were measured in the blood. Hyperemia and micro hemorrhages were examined by histopathological analysis. In addition, TNF-α, C-reactive protein (CRP), IL-1β, heat shock protein-70 (HSP70), and MDA expressions were evaluated. Results: The levels of MDA, BUN, Creatinine, and uric acid were increased, whereas CAT and SOD levels were decreased in LPS group. Histopathological and immunohistochemical examinations of the kidney tissue revealed hyperemia and micro hemorrhages together with an increase in TNF-α, CRP, IL-1β, HSP70, and MDA expressions in LPS group. Pretreatment with LCM prevented the development of all the pathological findings parameters in the kidney and serum. Conclusion: LCM pretreatment decreased oxidative damage in sepsis-induced kidney injury in the experimental model. However, LCM demonstrated favorable properties against sepsis-induced acute kidney injury reducing pro-inflammatory cytokine release.
Keywords
Lacosamide, Lipopolysaccharide, Kidney injury, Oxidative stress, Inflammation
Introduction
Sepsis is the extreme immune response of the body to microbial infections. It is defined as a life-threatening organ dysfunction. According to hospital records, the mortality rate is high all over the world. There are more than 31 million sepsis cases and 5 million sepsis-related deaths recorded each year [1,2]. Today, despite the usage of more effective pharmacological agents following a better understanding of the pathophysiology of sepsis, the cost of treatment continues to be an economic burden for many countries [3,4]. Recent studies have reported a decrease in sepsis-related mortality in intensive care units. However, these data are difficult to interpret as there may be differences in levels of countries’ development and pre-hospital and in-hospital response time and treatment [5]. Various epidemiological studies have pointed out that there is still no standard definition for sepsis. This hinders the comparability of sepsis outcomes worldwide [2,5]. Sepsis is recognized as a multiple organ dysfunction syndrome. Infections and non-infectious stimuli induce the release of significant amounts of cytokines by stimulating the innate immune system which results in sepsis [6]. Thus, sepsis is characterized by large cytokine release resulting in oxidative stress, mitochondrial dysfunction, cellular injury, and organ insufficiency [7].
50% of mortality rate in patients with sepsis, especially in intensive care units, is associated with acute kidney injury [8]. Our understanding of the pathogenesis of by sepsis-induced of acute kidney injury is limited, but it is now clear that septic acute kidney injury is different from ischemic acute kidney injury which has been identified and studied in a variety of experimental and clinical settings [9]. In early experimental studies of septic acute kidney injury, renal blood flow was reported to decline after the administration of endotoxin. The results of these endotoxin-based experiments led to the conclusion that septic acute kidney injury in humans may result from kidney vasoconstriction and ischemia [10]. The diagnosis of acute kidney injury is based on a sudden decrease in glomerular filtration rate and increase in serum Creatinine level [11]. The pathophysiology of sepsis-associated acute kidney injury is characterized by a decreased glomerular filtration rate (GFR), deterioration in peritubular capillary circulation, systemic and local inflammatory cytokine release, and tubular dysfunction caused by oxidative stress [12].
Information on sepsis-induced renal tubular dysfunction is insufficient. However, reduction in glomerular filtration rate of toxic blood is thought to cause tubular cell damage and oxidative stress [13]. Thus, cytokines, chemokine’s, complement fragments, and other cellular components in sepsis have a toxic effect on the luminal surface of tubular cells. This "Inflammatory theory of acute kidney injury" is supported by experimental studies. For example, lipopolysaccharide can interact with Toll-like receptors (TLR) expressed on tubular cells, and in response to inflammation, a decrease in energy consumption, autophagy, mitochondrial dysfunction, and loss of cell polarity may occur in the kidney [14]. In human and animal studies, it has been shown that the main characteristic of acute kidney injury caused by sepsis is not apoptosis, but inflammation; diffuse microcirculatory flow abnormalities, and cellular bioenergetics responses to injury [15].
Lacosamide (LCM) is an antiepileptic agent which is widely used in the adjunctive treatment of partial-onset seizures [16]. The properties of LCM include dissolution in water, complete absorption after oral administration, high oral bioavailability (100%), maximum plasma concentrations reached 0.5-4 h after intake [17], and passage across the blood-brain barrier [18]. LCM is mainly eliminated by renal excretion. LCM selectively enhances slow inactivation of voltage-gated sodium channels, unlike traditional sodium channel blockers [17]. In experimental models, LCM has been shown to inhibit inflammation and lipid peroxidation with its antioxidant and anti-inflammatory activities [19].
Investigating the effects of lacosamide on sepsis may lead to a new roadmap in sepsis treatment. The purpose of this study was to investigate the anti-inflammatory and antioxidative effects of lacosamide on sepsis-induced acute kidney injury.
Materials and Methods
Animals
Twenty-four female Wistar albino rats weighing 300-350 g were used in this study. Environmental conditions for the laboratory animals during the experiment were established as follows: ad libitum feeding, tap water, 12-h light/dark cycle, temperature 22 ± 2ºC, and humidity 55- 60%. The experimental procedures were approved by the Animal Experiments Local Ethics Committee of Mehmet Akif Ersoy University (Ethic No: 543, 18/09/2019). The study was conducted in accordance with the animal research guidelines of the National Institute of Health. The maintenance, housing, and feeding conditions of the animals were the same in all groups.
Study groups
Twenty-four female Wistar-albino rats were randomly divided into three groups (n=8):
Control group: rats received of daily administration of normal saline via intraperitoneal (i.p.) injection for 3 days.
LPS group: rats received a single dose of LPS (5 mg/ kg, i.p) and normal saline (i.p., for 3 days before LPS application). LPS (Sigma-Aldrich, Steinheim am Albuch, Germany) was dissolved in normal saline. The dose of LPS used in the study was based on previous studies in which rats were shown to experience endotoxemia after the administration of 5 mg/kg LPS [15,20]. LPS-induced systemic inflammation has been a widely used model for sepsis studies [21].
LPS+LCM group: rats received a single dose of LPS (5 mg/kg, i.p) and LCM (40 mg/kg, i.p., for 3 days before LPS application). LCM (Adeka Farmacy, Turkey) was dissolved in normal saline. LPS was injected 30 minutes after the last LCM application. The dose of LCM used in the study was based on previous studies [19]. Renewed current pharmacokinetics of LCM was taken into account in determining the LCM treatment.
Six hours after the last LPS administration [19,20] all rats were sacrificed via ketamine-xylazine anesthesia (90 mg/kg ketamine (Alfamine, Alfasan IBV) and 10 mg/ kg xylazine (Alfazin, Alfasan IBV).
After abdominal incision, blood samples from all animals were collected from inferior vena cava. The obtained blood samples were centrifuged at 10000g for 15 min at 4oC. The collected serum was stored at -80oC for biochemical analysis. At the same time, bilateral nephrectomy was performed. Right kidneys were placed in 10% formaldehyde solution for histopathological examinations. Left kidneys were homogenized and stored at -80°C for biochemical analysis.
Biochemical analysis
Preparation of tissue samples
The kidney tissues obtained from each animal were placed in phosphate buffer (pH 7.4) and shredded with a homogenizer (IKA Ultra-Turrax T25 Basic; Labortechnic, Staufen, Germany) and a sonicator (UW- 2070 Bandelin Electronic, Germany). Then, the samples were centrifuged at 4000 rpm for 10 min at +4°C. Tissue samples were stored at -80°C for biochemical analysis.
Assay of malondialdehyde (MDA)
The concentration of protein was defined by the Bradford method [22]. Draper and Hadley's (1990) double heating method was used for MDA determination [23]. The samples were incubated with thiobarbituric acid, and the absorbance of the resulting colored complex was measured spectrophotometrically at 532 nm against a blank sample. The result was presented as μmol/mg protein.
Assay of superoxide dismutase (SOD)
SOD enzyme activity was analyzed according to the method of Woolliams et al. This method employs xanthine and xanthine oxidase to generate superoxide radicals which react with 2-(4-iodophenyl)-3-(4-nitrophenol)-5- phenyltetrazolium chloride (INT) to form a red formazan dye. The resulting change in absorbance was read at 560 nm using a spectrophotometer and presented as U/mg protein [24].
Assay of catalase (CAT)
CAT activity was evaluated using the Aebi method. Hydrogen peroxide was used as a reaction substrate, and the absorbance was determined spectrophotometrically at 240 nm [25].
Assay of BUN, Creatinine, and uric acid levels in serum
To assess renal functions, serum BUN, Creatinine, and uric acid levels were analyzed using Olympus Auto Analyzer (Olympus Instruments, Tokyo, Japan). The results were presented as mg/dl.
Histopathological analyses
At necropsy, the kidneys were macroscopically evaluated, and tissue samples were collected. The collected kidney tissues were fixed in a buffered 10% neutral formalin solution and routinely processed by a tissue processor (Leica ASP300S automated tissue processor, Wetzlar, Germany). Subsequently, the samples were embedded in paraffin. Then, the sections at 5-μm thickness were cut from the paraffin blocks by a fully motorized rotary microtome (Leica RM2155, Leica Microsystems, Wetzlar Germany). Subsequently, the sections were routinely stained with hematoxylin and eosin (Harris staining method) and evaluated under a light microscope. The microscopic findings were evaluated and blindly graded, and the lesions were examined by an independent pathologist. Histopathological changes were evaluated for three parameters: (a) hemorrhage, (b) edema, and (c) hyperemia. For damage rating, no damage (-), mild damage (+), moderate damage (++), severe damage (+++) were used.
Immunohistochemical analyses
The kidney samples were immnuostained with interleukin-1β [Anti-IL1 beta antibody (ab2105)], MDA [Anti-Malondialdehyde antibody (ab6463)], heat shock protein-70 [Anti-Hsp70 antibody [5A5] (ab2787)], C-reactive protein [Anti-C Reactive Protein antibody- Aminoterminal end (ab65842)], and Tumor necrosis factor-alpha [Anti-TNF alpha antibody (ab6671)] by streptavidin-biotin method. IHC kits and all primary serums were purchased from Abcam (Cambridge, UK). The sections were incubated with primary antibodies for 60 minutes. Then, the sections were incubated with biotinylated secondary antibody and streptavidinalkaline phosphatase conjugate. EXPOSE Mouse and Rabbit Specific HRP/DAB Detection IHC kit (ab80436) was used for the secondary antibody. Diaminobenzidine (DAB) was used as the chromogen. Positive and negative controls were performed for each marker. For negative controls, the primary antiserum step was omitted. All immunohistochemical examinations were performed in a blinded manner by a pathologist who was unaware of the groups. To evaluate the percentage of positively immunostained cells for each marker, the cells from each group were counted in 10 different areas in every section at X40 magnification. All the slides were analyzed for immunopositivity, and a semiquantitative analysis was conducted. The samples were scored from 0 to 3 according to the intensity of staining (0=absence of staining; 1=slight, 2=medium, and 3=marked staining). The groups’ scores were statistically analyzed to evaluate differences between the groups. Morphometric examinations were performed using the Database Manual CellSens Life Science Imaging Software (Olympus Co., Tokyo, Japan).
Statistical analysis
Statistical analysis of the data was done using SPSS 22.0 for Windows. The data were tested for the normality of distribution by Kolmogorov Smirnov and Shapiro- Wilk tests. All the data showed normal distribution, thus, one-way ANOVA and post hoc LSD tests were used to compare biochemical, histopathological, and immunohistochemical scores of the groups. Variables were presented as mean ± standard deviations. P<0.05 was considered statistically significant.
Results
Biochemical results
Effects of LCM on malondialdehyde in LPS-induced kidney injury
LPS administration caused a significant increase in MDA levels compared to the control group (p=0.001). LCM treatment significantly decreased MDA levels compared to LPS group (p=0.001) (Figure 1).
Effects of LCM on catalase and superoxide dismutase enzyme activity in LPS-induced kidney injury
In LPS group, CAT and SOD activity levels were significantly decreased compared to the control group (p=0.001 and p=0.037, respectively). However, LCM administration significantly increased CAT and SOD activity levels compared to LPS group (p=0.005 and p=0.004, respectively). All the results are shown in Figure 1.
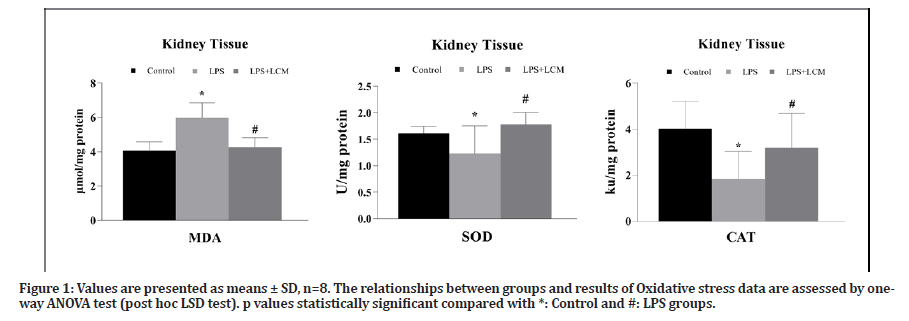
Figure 1: Values are presented as means ± SD, n=8. The relationships between groups and results of Oxidative stress data are assessed by oneway ANOVA test (post hoc LSD test). p values statistically significant compared with *: Control and #: LPS groups.
Effects of LCM on serum creatinine, BUN, and uric acid levels in LPS-induced kidney injury
BUN, creatinine, and uric acid levels of all groups are shown in Table 1. BUN levels were increased in LPS group compared to the control group (p=0.001). LCM administration significantly reduced BUN levels in LPS+LCM group compared to LPS group (p=0.004). In LPS group, creatinine levels were significantly increased compared to the control group (p=0.001). Creatinine levels did not decrease significantly in LPS + LCM group compared to LPS group. Uric acid levels in LPS group were increased compared to the control group (p=0.004). LCM treatment significantly decreased uric acid levels compared to LPS group (p=0.006).
Groups | BUN (mg/dL) | Creatinine (mg/dL) | Uric Acid (mg/dl) | |||
---|---|---|---|---|---|---|
Mean ± SD | P value | Mean ± SD | P value | Mean ± SD | P value | |
Control | 19.75 ± 1.66 | 0.43 ± 0.05 | 1.03 ± 0.31 | |||
LPS | 35.66 ± 7.08 | a0.001 | 0.59 ± 0.09 | a0.001 | 1.73 ± 0.54 | a0.004 |
LPS+LCM | 24.66 ± 7.50 | b0.004 | 0.58 ± 0.08 | 1.03 ± 0.28 | b0.006 | |
Values are presented as means ± SD, n=8. The relationships between groups and results of BUN, creatinine and uric acid levels are assessed by one-way ANOVA test (post hoc LSD test). p values statistically significant compared with a: control and b: LPS groups. BUN (Blood urea nitrogen) |
Table 1: Serum creatinine, BUN and uric acid data of all groups.
Histopathological findings
At microscopic examination, no pathological findings were observed in the kidney of the control group (grade 0). Following LPS exposure (LPS group), marked hyperemia, edema, and scattered hemorrhagic areas were observed (grade +++). However, LCM treatment (LPS+LCM group) ameliorated these histopathological findings (grade - ) (Figure 2).
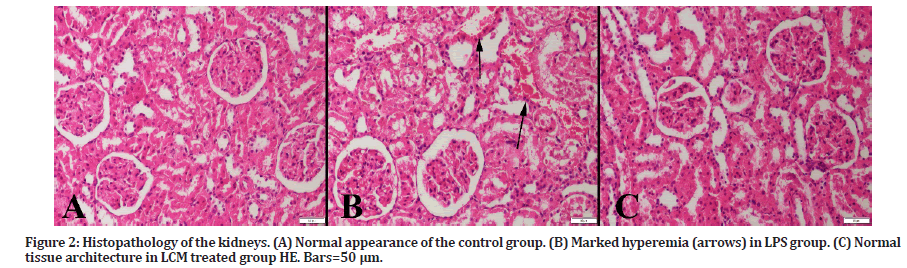
Figure 2: Histopathology of the kidneys. (A) Normal appearance of the control group. (B) Marked hyperemia (arrows) in LPS group. (C) Normal tissue architecture in LCM treated group HE. Bars=50 μm.
Immunohistochemical findings
A marked increase in TNF-α expression was observed in tubular and glomerular cells in LPS group compared to the control group (p<0.001). LCM treatment decreased the number of TNF-α positive cells in LPS+LCM group compared to LPS group (p<0.001) (Figure 3). We found an increased IL-1β expression in tubular cells in LPS group compared to the control group (p<0.001), while no expression was found in other groups (Figure 4). A marked increase in CRP expression was observed in tubular and glomerular cells in LPS group compared to the control group (p<0.001). LCM treatment slightly ameliorated CRP values in LPS+LCM group compared to LPS group (Figure 5). We found an increased HSP70 expression in tubular cells of LPS group compared to the control group (p<0.001). LCM treatment decreased HSP70 expression in LPS+LCM group compared to LPS group (p<0.001) (Figure 6). We found an increased MDA expression in LPS group compared to the control group (p<0.001) in tubular and glomerular cells. LCM treatment decreased the number of these cells in LPS+LCM group compared to LPS group (Figure 7). The results of the statistical analysis of immuno-histochemically positive cells are shown in Figures 3-7.
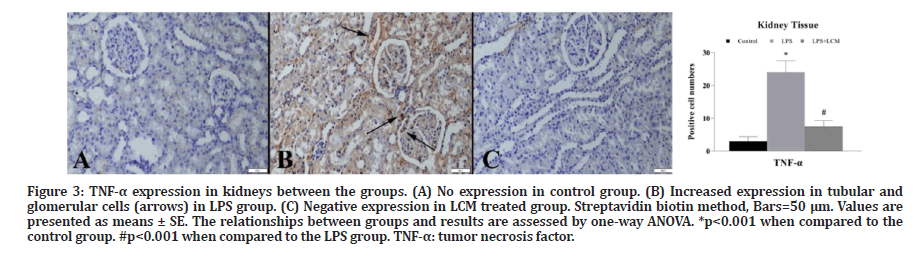
Figure 3: TNF-μ expression in kidneys between the groups. (A) No expression in control group. (B) Increased expression in tubular and glomerular cells (arrows) in LPS group. (C) Negative expression in LCM treated group. Streptavidin biotin method, Bars=50 μm. Values are presented as means ± SE. The relationships between groups and results are assessed by one-way ANOVA. *p<0.001 when compared to the control group. #p<0.001 when compared to the LPS group. TNF-μ: tumor necrosis factor.
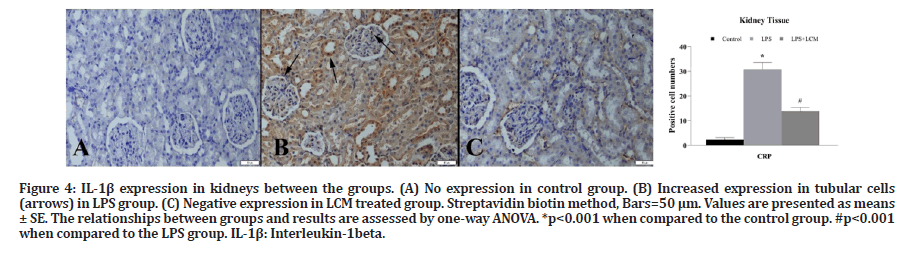
Figure 4:IL-1β expression in kidneys between the groups. (A) No expression in control group. (B) Increased expression in tubular cells (arrows) in LPS group. (C) Negative expression in LCM treated group. Streptavidin biotin method, Bars=50 μm. Values are presented as means± SE. The relationships between groups and results are assessed by one-way ANOVA. *p<0.001 when compared to the control group. #p<0.001 when compared to the LPS group. IL-1β: Interleukin-1beta.
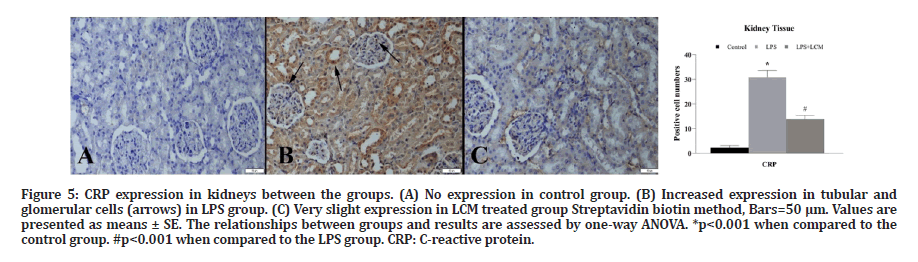
Figure 5: CRP expression in kidneys between the groups. (A) No expression in control group. (B) Increased expression in tubular and glomerular cells (arrows) in LPS group. (C) Very slight expression in LCM treated group Streptavidin biotin method, Bars=50 μm. Values are presented as means ± SE. The relationships between groups and results are assessed by one-way ANOVA. *p<0.001 when compared to the control group. #p<0.001 when compared to the LPS group. CRP: C-reactive protein.
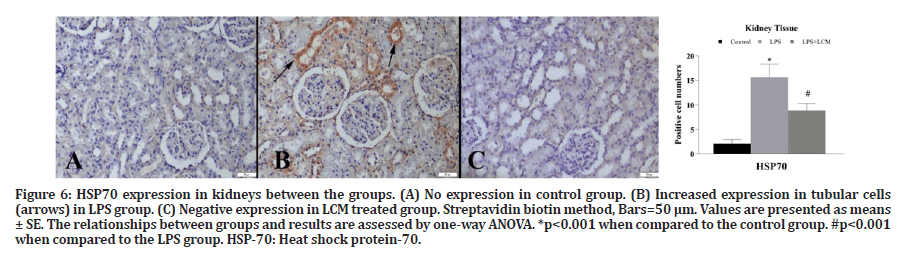
Figure 6: HSP70 expression in kidneys between the groups. (A) No expression in control group. (B) Increased expression in tubular cells (arrows) in LPS group. (C) Negative expression in LCM treated group. Streptavidin biotin method, Bars=50 μm. Values are presented as means± SE. The relationships between groups and results are assessed by one-way ANOVA. *p<0.001 when compared to the control group. #p<0.001 when compared to the LPS group. HSP-70: Heat shock protein-70.
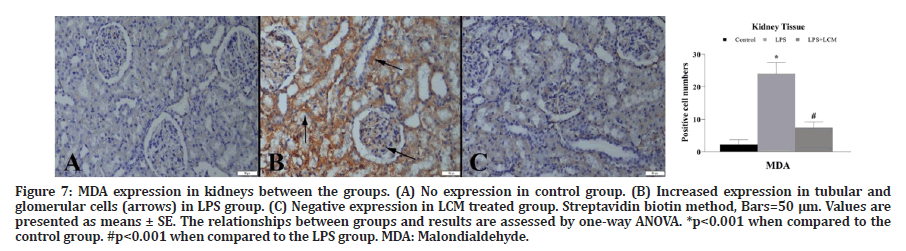
Figure 7: MDA expression in kidneys between the groups. (A) No expression in control group. (B) Increased expression in tubular and glomerular cells (arrows) in LPS group. (C) Negative expression in LCM treated group. Streptavidin biotin method, Bars=50 μm. Values are presented as means ± SE. The relationships between groups and results are assessed by one-way ANOVA. *p<0.001 when compared to the
control group. #p<0.001 when compared to the LPS group. MDA: Malondialdehyde.
Discussion
As we demonstrated in this experimental study, LCM has therapeutic effects on lipopolysaccharide-induced kidney injury in rats. Oxidative stress caused by LPS leads to free radical formation and subsequent protein oxidation, lipid peroxidation, and DNA damage leading to the tissue injury.
Oxidative stress and mitochondrial dysfunction are thought to play an important role in the development of sepsis-induced kidney injury [26]. In a recent study, researchers investigated the indicators of oxidative stress and mitochondrial dysfunction in an in vitro model of sepsis-induced renal injury. They demonstrated that LPS application increased the expressions of NADPH oxidase 4, nitric oxide, superoxide anion, reactive nitrogen species (RNS), and reactive oxygen species (ROS) [27]. In another study, researchers examined the role of endogenous and exogenous ROS scavengers to evaluate the potential impact of ROS on acute renal injury associated with endotoxemia. The results of the study showed that renal vasoconstriction during endotoxemia involve a down regulation of renal extracellular SOD by reduction of NO function by ROS. In another research, the effect of Honokiol (Houpo) on acute kidney injury in cecal ligation and puncture (CLP)-induced sepsis model was investigated, and it was shown that SOD activity was reduced in the sepsis group [28]. Malondialdehyde (MDA) is a major and most thoroughly researched oxidation product of peroxidized polyunsaturated fatty acids. It is an important indicator of oxidative stress [29]. In recent studies, MDA has been used as an indicator of many diseases such as cancer [30], diabetes [31], neurodegenerative diseases [32], cardiovascular diseases, liver and kidney diseases [29]. In our study, the activity of MDA, the oxidative stress marker, was increased, and SOD and CAT activities were decreased in the kidney tissues of animals exposed to LPS, supporting the previous data that showed that LPS induced kidney injury. According to our findings, LCM treatment ameliorated these parameters.
Antioxidant therapy plays an important role in regulating mitochondrial membrane polarization required for oxidative phosphorylation in acute kidney injury caused by sepsis [33]. Kumar et al. investigated the pharmacokinetic and pharmacodynamics properties of lacosamide in experimentally induced hepatic and renal impairment in seizure animals. They showed that LCM (25 mg kg−1) decreased MDA levels in the kidney and increased SOD and CAT enzyme activities [34]. In our study, a significant decrease in MDA levels and an increase in SOD and CAT activities with LCM treatment showed that kidney injury caused by LPS-stimulated oxidative stress in rats can be prevented. Further studies are needed to elaborate on the effects of LCM therapy on the kidney injury caused by oxidative stress due to sepsis. It will contribute to a limited number of studies in this field.
BUN and serum creatinine are important biomarkers indicating acute kidney injury. However, serum creatinine concentrations may be increased by other non-renal factors such as muscle mass, liver function, and gastrointestinal elimination [35]. In several previous studies, it was shown that serum creatinine levels significantly increased 6 hours after CLP and LPS administration in the sepsis model [36-39]. Similar to these studies, our study findings revealed that serum creatinine, BUN, and uric acid levels significantly increased after LPS administration. LCM slightly ameliorated BUN and uric acid levels. Non-significant decrease in serum creatinine levels after LCM treatment was probably due to the short treatment duration in the experimental design. Further studies should evaluate whether longer treatment duration will be more effective.
Changes in vascular permeability are thought to be a key factor in the pathogenesis of sepsis-induced organ failure. An increased vascular permeability can lead to an increased peritubular capillary permeability, reduced microvascular flow, and hemorrhage [40]. Yasuda et al. reported renal capillary leakage 6 hours after CLP administration [36]. Wang Z et al., (2012) demonstrated that capillary leakage occurs as early as 2 hours after CLP [33]. They also showed a decreased renal blood flow and peritubular capillary perfusion. Our data demonstrate that hyperemia and micro hemorrhages occur 6 hours after LPS administration. The results of histopathological examination of the kidney tissue of animals receiving LCM pretreatment were similar to the ones in the control group. According to our results, we can affirm that LCM pretreatment improves the findings of micro hemorrhage and hyperemia in the kidney.
Inflammation is thought to play an important role in the pathophysiology of acute kidney injury. In the present study, we investigated the production of TNF-alpha and IL-1 proinflammatory cytokines in the kidney. TNFalpha is an important indicator of acute renal injury [41]. In the study, TNF-α was reported to be a main indicator of inflammatory and hemodynamic responses caused by sepsis-induced acute kidney injury and release of other cytokines [39]. IL-1β cytokine is a critical component of the inflammation process. In a study reporting that betulin reduced kidney injury in septic rats by inhibiting TLR4 / NF-κB signaling pathway, researchers demonstrated that TNF-α, IL-1β, and IL-6 secretion drastically increased after LPS treatment [41]. Gu et al., in line with the previous studies, indicated that plasma TNF-α, IL-1β, and IL-10 expressions were increased 4 hours after LPS exposure. Similar to the previous results, we found that LPS increased the secretion of TNF-α and IL-1β in the kidney [42].
CRP is commonly used as a marker of an acute inflammatory state [43]. It is widely used in the clinic for the diagnosis of sepsis. High serum CRP concentrations are associated with the risk of organ failure and death, and monitoring CRP levels through the course of disease can help assess its response to the treatment in septic patients [44]. In a study investigating the relationship between CRP and leptin levels in septic men, it was shown that CRP, TNF-α, IL-1β, and IL-6 expressions were significantly elevated in the septic group [45]. CRP is the main acute phase reactant synthesized in the liver in humans. However, Jabs et al. showed that CRP may be synthesized in extrahepatic organs in case of inflammation. In their study, researchers demonstrated the formation of CRP in cortical tubular cells in vivo [46]. Similarly, we demonstrated an increase in CRP secretion in the kidney 6 hours after LPS administration by immunohistochemistry.
Elevated HSPs expressions are known to be caused by various pathological conditions such as temperature rise, oxidative stress, and inflammation [47]. In numerous studies it has been shown that HSP70 was able to reduce the release of proinflammatory cytokines and decrease apoptosis in renal and lung cells. Thus, it has been shown that HSP70 can regulate cytokine release [48]. Therefore, in addition to TNF-α, IL-1β, and CRP inflammatory mediators, we investigated the relationship between HSP70 expression, sepsis, and acute renal injury. Our results showed an increased HSP70 expression in acute kidney injury induced by sepsis.
Lacosamide is a potential therapeutic agent for sepsisinduced acute kidney injury. Some cytokines (such as TNF-α, IL-1β) that stimulate inflammatory reactions are activated in response to oxidative stress [49]. In a previous study, researchers demonstrated that LCM significantly reduced TNF-α, IL-1β, and IL-6 levels in brain, liver, and kidney injuries in an experimental epilepsy model [29]. In another study investigating potential anti-inflammatory and antioxidant effects of LCM, researchers examined TNF-α, CRP, and MDA levels in rats with sepsis-induced neuropathy. They showed that LCM significantly reduced TNF-α, CRP, and MDA levels compared to the sepsis group [16]. We found that LCM inhibited TNF-α, IL-1β, CRP, and HSP70 elevation in the kidney 6 hours after LPS administration (Figures 3-7). However, further research is needed to clarify these results.
There is limited research on the anti-inflammatory effect of lacosamide. However, lacosamide has been identified as a good alternative in diseases as well as epilepsy in recent studies, it has been shown to have neuroprotective properties with an effective reduction in neuroinflammation and improvement signals in neurogenesis. Promising results have been found in in vitro and in vivo models [50]. In line with these promising studies, we investigated that LCM may have antiinflammatory effects on kidney tissue. In our study, we used LPS to induce acute kidney injury and prophylactic LCM treatment improved TNF-α, IL-1β, CRP, HSP70 and MDA expressions caused by LPS.
Conclusion
In conclusion, it should be noted that this is the first study demonstrating anti-inflammatory and antioxidant effects of lacosamide pretreatment on LPS-induced acute kidney injury in rats. LCM showed favorable effects on acute kidney injury by decreasing MDA levels and increasing SOD and CAT enzyme activities. In addition, LCM decreased TNF-α, IL-1, CRP, and HSP70 expressions in LPS-induced acute kidney injury in rats. Overall, on the basis of the data presented here, LCM may be useful not only as an anti-epileptic, but also in the case of sepsis occurring in acute kidney injury. However, in vitro studies in futures would help to describe the effect of lacosamide on immune cells and tubule cells. Also, It is important to study the efficacy of LCM in different populations.
References
- Walkey AJ, Lagu T, Lindenauer PK. Trends in sepsis and infection sources in the United States. A population-based study. Ann Am Thorac Soc 2015; 12:216–220.
- Fleischmann C, Scherag A, Adhikari NKJ, et al. Assessment of global incidence and mortality of hospital-treated sepsis: Current estimates and limitations. Am J Respir Crit Care Med 2016; 193:259-272.
- Cohen J, Vincent JL, Adhikari NK, et al. Sepsis: A roadmap for future research. Lancet Infect Dis 2015; 15:581–614.
- Seymour CW, Liu VX, Iwashyn TJ, et al. Assessment of clinical criteria for sepsis for the third international consensus definitions for sepsis and septic shock (Sepsis-3). JAMA 2016; 315:762-774.
- Shankar-Hari M, Harrison DA, Rowan KM. Differences in impact of definitional elements on mortality precludes international comparisons of sepsis epidemiology—A cohort study illustrating the need for standardized reporting. Crit Care Med 2016; 44:2223–2230.
- Vincet JL, Opal SM, Marshall JC, et al. Sepsis definitions: Time for change. Lancet 2013; 381:774–775.
- Ren C, Tong YL, Li JC, et al. Early antagonism of cerebral high mobility group box-1 protein is benefit for sepsis induced brain injury. Oncotarget 2017; 8:92578–92588.
- Zarjou A, Agarwal A. Sepsis and acute kidney injury. J Am Soc Nephrol 2011; 22:999 –1006.
- Gomez H, Ince C, Backer DD, et al. A unified theory of sepsis-induced acute kidney injury: inflammation, microcirculatory dysfunction, bioenergetics and the tubular cell adaptation to injury. Shock 2014; 41:3–11.
- Schrier RW, Wang W. Acute renal failure and sepsis. N Engl J Med 2004; 351:159–169.
- Doi K, Leelahavanichkul A, Yuen PST, et al. Animal models of sepsis and sepsis-induced kidney injury. J Clin Inves 2009a; 119:2868–2878.
- Wu L, Gokden N, Mayeux PR. Evidence for the role of reactive nitrogen species in polymicrobial sepsis-induced renal peritubular capillary dysfunction and tubular injury. J Am Soc Nephrol 2007; 18:1807–1815.
- Mårtensson J, Bellomo R. Sepsis-induced acute kidney injury. Crit Care Clin 2015; 31:649-660.
- Bellomo R, Kellum JA, Ronco C, et al. Acute kidney injury in sepsis. Intensive Care Med 2017; 43:816–828.
- Kiyonaga N, Moriyama T, Kanmura Y. Effects of landiolol in lipopolysaccharide-induced acute kidney injury in rats and in vitro. Shock 2019; 52:e117–e123.
- Choi HY, Park JH, Chen BH, et al. Increases of catalase and glutathione peroxidase expressions by lacosamide pretreatment contributes to neuroprotection against experimentally induced transient cerebral ischemia. Neurochem Res 2016; 41:2380–2390.
- Diaz A, Deliz B, Benbadis SR. The use of newer antiepileptic drugs in patients with renal failure. Expert Rev Neurother 2012; 12:99–105.
- Cawello W. Clinical pharmacokinetic and pharmacodynamic profile of lacosamide. Clin Pharmacokinet 2015; 54:901–914.
- Solmaz V, Aksoy D, Yılmaz M, et al. Demonstration of ameliorative effect of lacosamide: in a rat model of sepsis-induced critical illness polyneuropathy. Neurol Res 2015; 37:797-802.
- Ayaz G, Halici Z, Albayrak A, et al. Evaluation of 5-HT7 receptor trafficking on in vivo and in vitro model of lipopolysaccharide (LPS)-Induced inflammatory cell injury in rats and LPS-treated A549 cells. Biochem Genetics 2017; 55:34–47.
- Nozaki Y, Hino S, Ri J, et al. Lipopolysaccharide-induced acute kidney injury is dependent on an IL-18 receptor signaling pathway. Int J Mol Sci 2017; 18:2777.
- Bradford MM. A rapid and sensitive method for the quantitation of microgram quantities of protein utilizing the principle of protein-dye binding. Anal Biochem 1976; 72:248–254.
- Drapper HH, Hadley M. Malondialdehyde determination as index of lipid peroxidation. Methods Enzymol 1990; 186:421–431.
- Woolliams JA, Wiener G, Anderson PH, et al. Variation in the activities of glutathione peroxidase and superoxide dismutase and in the concentration of copper in the blood various breed crosses of sheep. Res Vet Sci 1983; 34:69–77.
- Aebi H. Catalase in vitro. Methods Enzymol 1984; 105:121–126.
- Wang W, Jittikanont S, Falk SA, et al. Interaction among nitric oxide, reactive oxygen species, and antioxidants during endotoxemia-related acute renal failure. Am J Physiol Renal Physiol 2003; 284:F532–F537.
- Quoilin C, Mouithys-Mickalad A, Lécart S, et al. Evidence of oxidative stress and mitochondrial respiratory chain dysfunction in an in vitro model of sepsis-induced kidney injury. Biochem Biophys Acta 2014; 1837:1790-1800.
- Li N, Xie H, Li L, Wang J, et al. Effects of honokiol on sepsis-induced acute kidney injury in an experimental model of sepsis in rats. Inflammation 2014; 37:1191–1199.
- Del Rio D, Stewart AJ, Pellegrini N. A review of recent studies on malondialdehydeas toxic molecule and biological marker of oxidative stress. Nutr Metab Cardiovasc Dis 2005; 15:316-328.
- Gönenç A, Özkan Y, Torun M, et al. Plasma malondialdehyde (MDA) levels in breast and lung cancer patients. J Clin Pharm Therapeutics 2001; 26:141-144.
- Martin-Gallan P, Carrascosa A, Gussinye M, et al. Biomarkers of diabetes-associated oxidative stress andantioxidant status in young diabetic patients with or without subclinical complications. Free Radic Biol Med 2003; 34:1563-1574.
- Dib M, Garrel C, Favier A, et al. Can malondialdehyde be used as a biological marker of progression in neurodegenerative disease? J Neurol 2002; 249:367–337.
- Wang Z, Holthoff JH, Seely KA, et al. Development of oxidative stress in the peritubular capillary microenvironment mediates sepsis-induced renal microcirculatory failure and acute kidney injury. Am J Pathol 2012; 180:505–516.
- Kumar B, Modi M, Saika B, et al. Evaluation of brain pharmacokinetic and neuropharmaco, dynamic attributes of an antiepileptic drug, lacosamide, in hepatic and renal impairment: Preclinical evidence. Chem Neurosci 2017; 8:1589−1597.
- Doi K, Yuen PST, Eisner C, et al. Reduced production of creatinine limits its use as marker of kidney injury in sepsis. J Am Soc Nephrol 2009b; 20:1217–1221.
- Yasuda H, Yuen PST, Hu X, et al. Simvastatin improves sepsis-induced mortality and acute kidney injury via renal vascular effects. Kidney Int 2006; 69:1535–1542.
- Yang QH, Liu DW, Long Y, et al. Acute renal failure during sepsis: Potential role of cell cycle regulation. J Infect 2009; 58:459-464.
- Han M, Liu M, Li Y, et al. Renal neutrophil gelatinase associated lipocalin expression in lipopolysaccharide-induced acute kidney injury in the rat. BMC Nephrol 2012; 13:1-10.
- Khowailed A, Younan SM, Ashour H, et al. Effects of ghrelin on sepsis-induced acute kidney injury: one step forward. Clin Exp Nephrol 2015; 19:419–426.
- Lee WL, Slutsky AS. Sepsis and endothelial permeability. N Engl J Med 2010; 363:689–691.
- Zhao H, Zheng Q, Hu X, et al. Betulin attenuates kidney injury in septic rats through inhibitingTLR4/NF-κB signaling pathway. Life Sci 2016; 144:185-193.
- Gu G, Zhang Z, Wang G, et al. Effects of electroacupuncture pretreatment on inflammatory response and acute kidney injury in endotoxaemic rats. J Int Med Res 2011; 39:1783–1797.
- Aldo L, Enrico P, Romolo D, et al. Comparison of procalcitonin and C-reactive protein as markers of sepsis. Crit Care Med 2003; 31:1737-1741.
- Lobo SM, Lobo FR, Bota DP, et al. C-reactive protein levels correlate with mortality and organ failure in critically ill patients. Chest 2003; 123:2043-2049.
- Maruna P, Gurlich R, Frasko R, et al. Serum leptin levels in septic men correlate well with C-Reactive Protein (CRP) and TNF-alpha but not with BMI. Physiol Res 2001; 50:589-594.
- Jabs WJ, Lögering BA, Gerke P, et al. The kidney as a second site of human C-reactive protein formation in vivo. Eur J Immunol 2003; 33:152–161.
- Kim DJ, Park SH, Sheen MR, et al. Comparison of experimental lung injury from acute renal failure with injury due to sepsis. Respiration 2006; 73:815–824.
- Bruemmer-Smith S, Stüber F, Schroeder S. Protective functions of intracellular heat-shock protein (HSP) 70-expression in patients with severe sepsis. Intensive Care Med 2001; 27:1835–1841.
- Savran M, Ozmen O, Erzurumlu Y, et al. The impact of prophylactic lacosamide on lps-induced neuroinflammation in aged rats. Inflammation 2019; 42:1913-1924.
- Carona A, Bicker J, Silva R, et al. Pharmacology of lacosamide: From its molecular mechanisms and pharmacokinetics to future therapeutic applications. Life Sciences 2021; 275:119342.
Indexed at, Google Scholar, Cross Ref
Indexed at, Google Scholar, Cross Ref
Indexed at, Google Scholar, Cross Ref
Indexed at, Google Scholar, Cross Ref
Indexed at, Google Scholar, Cross Ref
Indexed at, Google Scholar, Cross Ref
Indexed at, Google Scholar, Cross Ref
Indexed at, Google Scholar, Cross Ref
Indexed at, Google Scholar, Cross Ref
Indexed at, Google Scholar, Cross Ref
Indexed at, Google Scholar, Cross Ref
Indexed at, Google Scholar, Cross Ref
Indexed at, Google Scholar, Cross Ref
Indexed at, Google Scholar, Cross Ref
Indexed at, Google Scholar, Cross Ref
Indexed at, Google Scholar, Cross Ref
Indexed at, Google Scholar, Cross Ref
Indexed at, Google Scholar, Cross Ref
Indexed at, Google Scholar, Cross Ref
Indexed at, Google Scholar, Cross Ref
Indexed at, Google Scholar, Cross Ref
Indexed at, Google Scholar, Cross Ref
Indexed at, Google Scholar, Cross Ref
Indexed at, Google Scholar, Cross Ref
Indexed at, Google Scholar, Cross Ref
Indexed at, Google Scholar, Cross Ref
Indexed at, Google Scholar, Cross Ref
Indexed at, Google Scholar, Cross Ref
Indexed at, Google Scholar, Cross Ref
Indexed at, Google Scholar, Cross Ref
Indexed at, Google Scholar, Cross Ref
Indexed at, Google Scholar, Cross Ref
Indexed at, Google Scholar, Cross Ref
Indexed at, Google Scholar, Cross Ref
Indexed at, Google Scholar, Cross Ref
Indexed at, Google Scholar, Cross Ref
Indexed at, Google Scholar, Cross Ref
Indexed at, Google Scholar, Cross Ref
Indexed at, Google Scholar, Cross Ref
Indexed at, Google Scholar, Cross Ref
Indexed at, Google Scholar, Cross Ref
Indexed at, Google Scholar, Cross Ref
Indexed at, Google Scholar, Cross Ref
Author Info
Rahime Aslankoc1, Ozlem Ozmen2, Oguzhan Kavrik1 and Arzu Yalcin1*
1Süleyman Demirel University, Faculty of Medicine, Department of Physiology, Isparta, Turkey2Department of Pathology, Faculty of Veterinary, Burdur Mehmet Akif Ersoy University, Burdur, Turkey
Citation: Rahime Aslankoc, Ozlem Ozmen, Oguzhan Kavrik, Arzu Yalcin, Oxidative Stress and Inflammation Markers in Lipopolysaccharide (LPS)-Induced Acute Kidney Injury in Rats–The Potential Role of Lacosamide, J Res Med Dent Sci, 2022, 10 (8):65-73.
Received: 03-Aug-2022, Manuscript No. jrmds-22-71091; , Pre QC No. jrmds-22-71091(PQ); Editor assigned: 18-Aug-2022, Pre QC No. jrmds-22-71091(PQ); Reviewed: 18-Aug-2022, QC No. jrmds-22-71091; Revised: 23-Aug-2022, Manuscript No. jrmds-22-71091(R); Published: 30-Aug-2022